Much of modern biology is built on addition and subtraction.
To interrogate a gene’s function, subtract it from the genome and watch what happens (often, death). To confirm a gene’s role in some disease, replace a defective copy with an unmutated version and see if the addition renders a fix.
Such simple arithmetic is fundamental to the natural sciences, says Karl Deisseroth, professor of bioengineering and a psychiatrist at Stanford University in California. “It is the foundation of not just genetics, but biochemistry and developmental biology.”
Past the level of a cell, however, that math gets exponentially more complex. The functions that interest neuroscientists depend on vast networks of neurons, calling for calculations across entire neural circuits.
Deisseroth and others developed the technique of optogenetics to do controlled experiments on neurons at this scale nearly 20 years ago. As its name suggests, the method uses pulses of light to activate genetically modified neurons that have channelrhodopsins, or opsins, dotted along their surface. These proteins shift their shape when struck by light, forming pores that usher sodium ions into the cell and trigger an action potential.
Neuroscientists have applied the tool to explore just about every behavior, “from social interaction to hunger, thirst, aggression, sleep, avoidance, anxiety and fear,” Deisseroth says, but it lacks precision for some experiments. Any neuron with an opsin will fire an action potential when struck by photons, which is good for studying larger brain regions but not for answering more nuanced questions about, as Deisseroth puts it, “how many neurons, of what kind, and with what relative action” underlie some condition.
A new technique called two-photon holographic optogenetics, developed only in the past few years, could change all that. Unlike traditional optogenetics, it stimulates individual neurons with much greater specificity. It can turn on or off hundreds of neurons at will, in almost any desired pattern. And it can mimic the natural rhythms of neuronal activity observed in mouse and, potentially, human brains, rendering previously unanswerable questions amenable to inquiry — including questions about autism.
Researchers have used standard optogenetics to characterize neural circuits that go awry in mouse models of Parkinson’s and Alzheimer’s disease, and to create interventions that can target and restore those circuits, potentially providing an alternative to pharmacological approaches. But studies of the neural circuits underlying autism — along with any possible interventions — have lagged behind.
“The problem is that there’s very few people working on circuits right now in autism,” says Carlos Portera-Cailliau, professor of neurology and neurobiology at the University of California, Los Angeles. “There’s a huge emphasis on molecular studies and genetic studies,” sometimes to the neglect of more systems-level investigations, he says, where a full account of autism is likely to lie.
That neglect may not be unwarranted. The equipment used to study neural circuits — especially the lasers and optical systems — are expensive and require specialized knowledge to operate, says Nicolas Pégard, assistant professor of computational biophotonics at the University of North Carolina at Chapel Hill.
Despite these barriers, researchers could use two-photon holographic optogenetics to stress test key theories about the condition’s origins, including the idea that it results from too much excitation in the brain. Mice with excess neuronal excitation shy away from an unfamiliar mouse, for instance, which researchers take as a proxy for the altered social behaviors seen in autistic people. If researchers could selectively trigger those excitatory neurons in mice to find the cells responsible, and then fine-tune the number of neurons involved, they might unveil the tipping point at which the animals’ brains favor social aversion.
In other words, researchers could conceivably “come to a more tractable, material, physical understanding of the core problem with autism,” Deisseroth says, “that might lead to better understanding and treatment.”
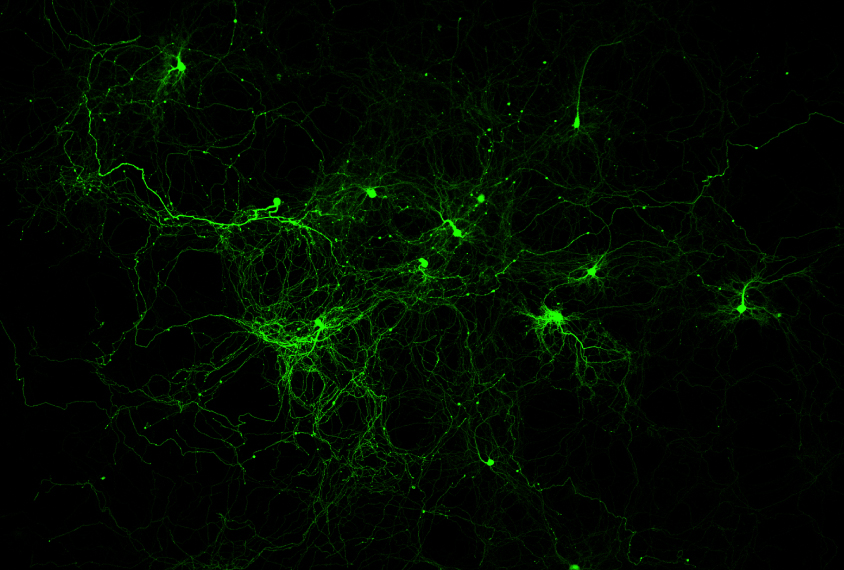
A
traditional optogenetics experiment goes something like this: Pack viruses with the gene that codes for an opsin and inject those viruses into mice. The viruses travel to the brain and dump their cargo into neurons. Genetic controllers ensure that the opsins are expressed in only a specific neuronal subtype, such as interneurons or GABAergic neurons.After a few days, drill a hole into the skull of each mouse and plug in an LED light. Voilà! With the flip of a switch, researchers activate the engineered neurons.
Two-photon holographic optogenetics improves upon these basic steps in several ways.
First, the opsin protein is modified to respond to infrared, rather than visible, light. Whereas visible light illuminates only the surface of tissue, near-infrared light penetrates a millimeter or more, which gives researchers control over neurons deeper in the brain. These infrared-sensing opsins trigger an action potential only when struck by two photons in quick succession (hence the term ‘two-photon’), ensuring that nearby neurons are not switched on accidentally.
Second, the virus is also packed with the gene for a calcium sensor protein, which binds to calcium ions and emits fluorescent light. Calcium rushes into neurons during action potentials and is a proxy for neuronal activity. Using both an opsin and calcium sensor protein makes it possible to simultaneously read and write neuronal information using two separate lasers: Infrared light at one wavelength triggers neurons to fire, whereas infrared light at another wavelength monitors fluorescence and records neuronal activity like a camcorder. Modern lasers can emit pulses 80 million times per second, fast enough to detect or trigger each action potential.
Third, the illumination technique allows for a depth dimension. Instead of wiring an LED into an animal’s brain, researchers swivel a microscope overhead and can zoom in on specific neurons, marking their location on a computer screen. From the neurons’ coordinates in 3D space, the system calculates a wave pattern that, as it propagates through the brain tissue, focuses energy at those locations.
To create this pattern, the system uses a spatial light modulator that consists of a liquid crystal, like those in a digital watch or old-school calculator. The crystals modify the wave phase of light impinging upon them. When the researchers shine a laser beam on the spatial light modulator, out comes the desired wave pattern. The laser beam is split, and each beamlet is redirected to a different neuron, like spotlights on a Broadway stage.
“Basically, it’s a fancy and expensive projector,” says Shai Berlin, assistant professor of neuroscience at Technion – Israel Institute of Technology in Haifa. “But instead of using small mirrors, we use very, very complicated physics and filters to modulate the phase of the light.”
In short, the approach generates an electronic hologram not unlike the 3D images in science-fiction movies. But instead of an image of, say, a rebel princess, this hologram is a 3D constellation of points of light that target specific locations in brain tissue. Two-photon holographic optogenetics, studies show, can monitor 3,000 neurons at once in 3D and activate a few hundred more. Rafael Yuste, professor of biology at Columbia University, equates this to “playing neurons like a piano.”
The technology is superior to conventional optogenetics because two neurons that lie next to each other in the brain may be genetically identical yet encode entirely distinct functions; it can tease those cells apart.
“With historical optogenetics, you would flood the brain with light and activate all the neurons that are genetically identical,” Pégard says, “which is kind of equivalent to hitting the person with a phone book on the head. You will get a reaction, but the level of control you have is pretty much all or nothing.”