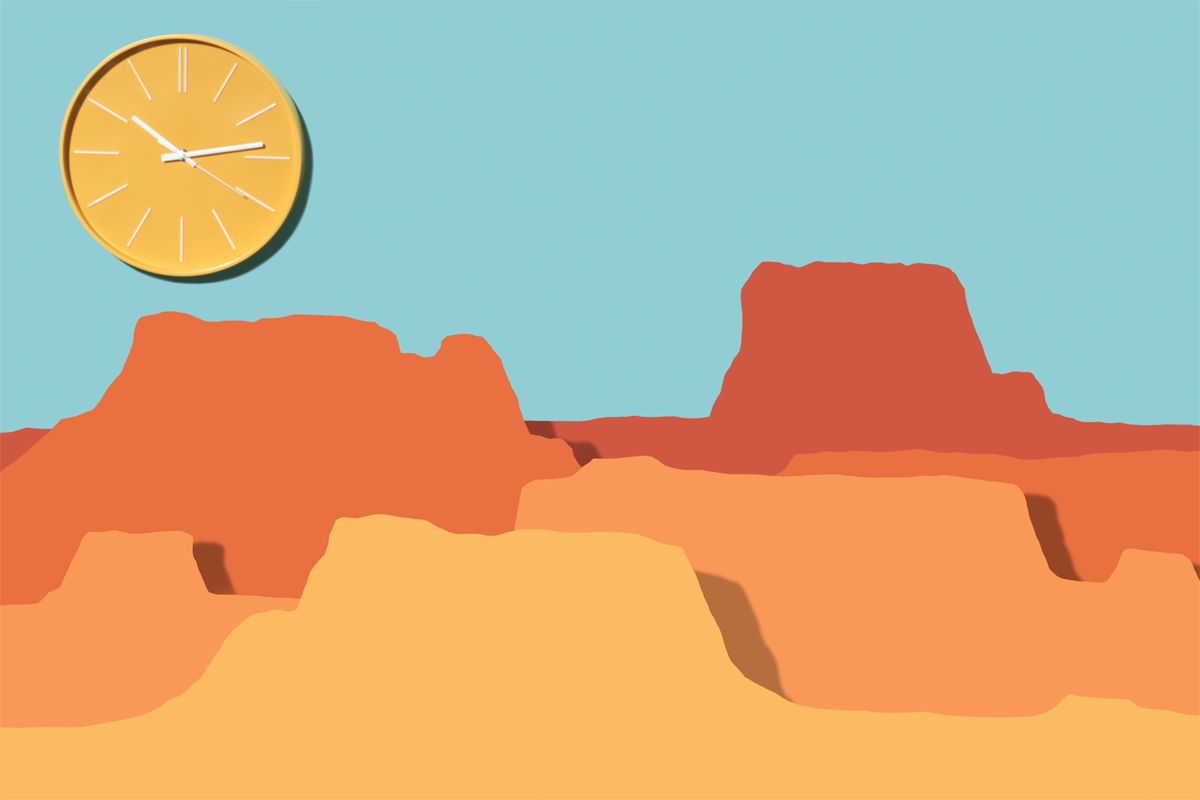
How to teach this paper: ‘Behavioral time scale synaptic plasticity underlies CA1 place fields,’ by Bittner and Milstein et al. (2017)
Katie Bittner, Aaron Milstein and their colleagues found that cellular learning can happen over longer timescales than Hebb’s rule predicts. How long should we wait to teach students about this phenomenon?
Most students in an introductory neuroscience class learn a catchy phrase to help them grasp Donald Hebb’s foundational idea that neuronal connections are strengthened when they are repeatedly active together: “Neurons that fire together, wire together.”
This so-called Hebbian plasticity has been shown to underlie many forms of memory, especially those formed when the brain associates cues and events within a narrow time frame—for example, the classic conditioning in Pavlov’s dogs, which learned to salivate at the sound of a bell that predicted dinner.
And yet Hebbian learning cannot be all there is, because not all stimuli and rewards are tightly locked in time. My dog readily hops into the car every morning because he knows that after a 20-minute drive to campus, open, manicured lawns and affectionate students await. Even though the rewards arrive later in time, my dog’s brain remembers the car as a (literal) vehicle for reward. There must be some neural mechanism to encode these kinds of associations that occur over longer periods of time.
In 2017, researchers working in Jeffrey Magee’s group (then at the Janelia Research Campus, now at Baylor College of Medicine) discovered a new form of neural plasticity that could account for some types of longer-term learning. Instead of requiring repetitive and simultaneous activation of cells within milliseconds, “behavioral time scale synaptic plasticity” (BTSP), as they described it in Science, can happen over seconds. It is likely that this type of plasticity underlies the kind of learning that happens over many seconds or with only a few repetitions. And remarkably, the authors were able to induce BTSP in mice with just one try—the kind of luck rarely encountered in science.
Paper overview
Friends, this Science paper doesn’t pull any punches—it throws you right into hippocampal dynamics and the limitations of Hebbian plasticity. The upshot is this: Single neurons in hippocampal area CA1 can rapidly “learn” to encode a specific location from a single surge of input several seconds before. This core message is presented in multiple forms, from modeling to in vitro and in vivo recordings. The paper covers a range of topics and could readily fit into courses covering introductory neuroscience, learning and memory, computational approaches or laboratory techniques. But before diving into the paper, students will need an overview of the common mechanisms of synaptic plasticity and hippocampal anatomy, as well as an introduction to dendritic calcium plateaus.
Pre-reading
The brain’s ability to learn and remember has long fascinated neuroscientists, who have sought molecular mechanisms of memory storage since the early days of our field. One of the primary forms of learning in the brain is through synaptic plasticity, or changes in synaptic connections tied to learning. Historically, synaptic plasticity research has centered on Hebb’s rule as a framework, looking for the molecular changes that occur when neurons fire together. Reviewing this history and some of the classic cellular mechanisms for synaptic plasticity (e.g., long-term potentiation) is a good place to start our discussion of this paper.
Hebbian learning has two big requirements that do not match all memory formation: Neurons must fire together on a very short timescale, and this must happen many, many times.
Magee’s group’s work on dendritic computations set the stage for their demonstration of BTSP. In 2015, they showed that properly timed input from hippocampal area CA3 and the entorhinal cortex onto a neuron in CA1 raises membrane potential in the dendrites, called a plateau. This transient depolarization causes the cell to fire a burst of action potentials. On subsequent trials, that same cell demonstrated a slow, ramping depolarization, suggesting that the strengths of its synaptic inputs had increased.
Given that increase, this ramping depolarization offers a possible mechanism for plasticity. But proof would require demonstration that the plateau influences the cell’s computation. To explore this, Magee’s team looked at plateaus’ relationship with hippocampal “place cells,” which fire selectively for particular locations in space. By recording from the CA1 cells while mice ran on a treadmill, they saw that the dendritic plateaus seemed to happen when the animal was in that neuron’s place field. Remarkably, plateaus often preceded abrupt place-field formation in previously silent neurons. To show that the plateau was vital for the formation of the place field, they needed to induce plateaus.
With those bases covered, you can dig into the paper itself.
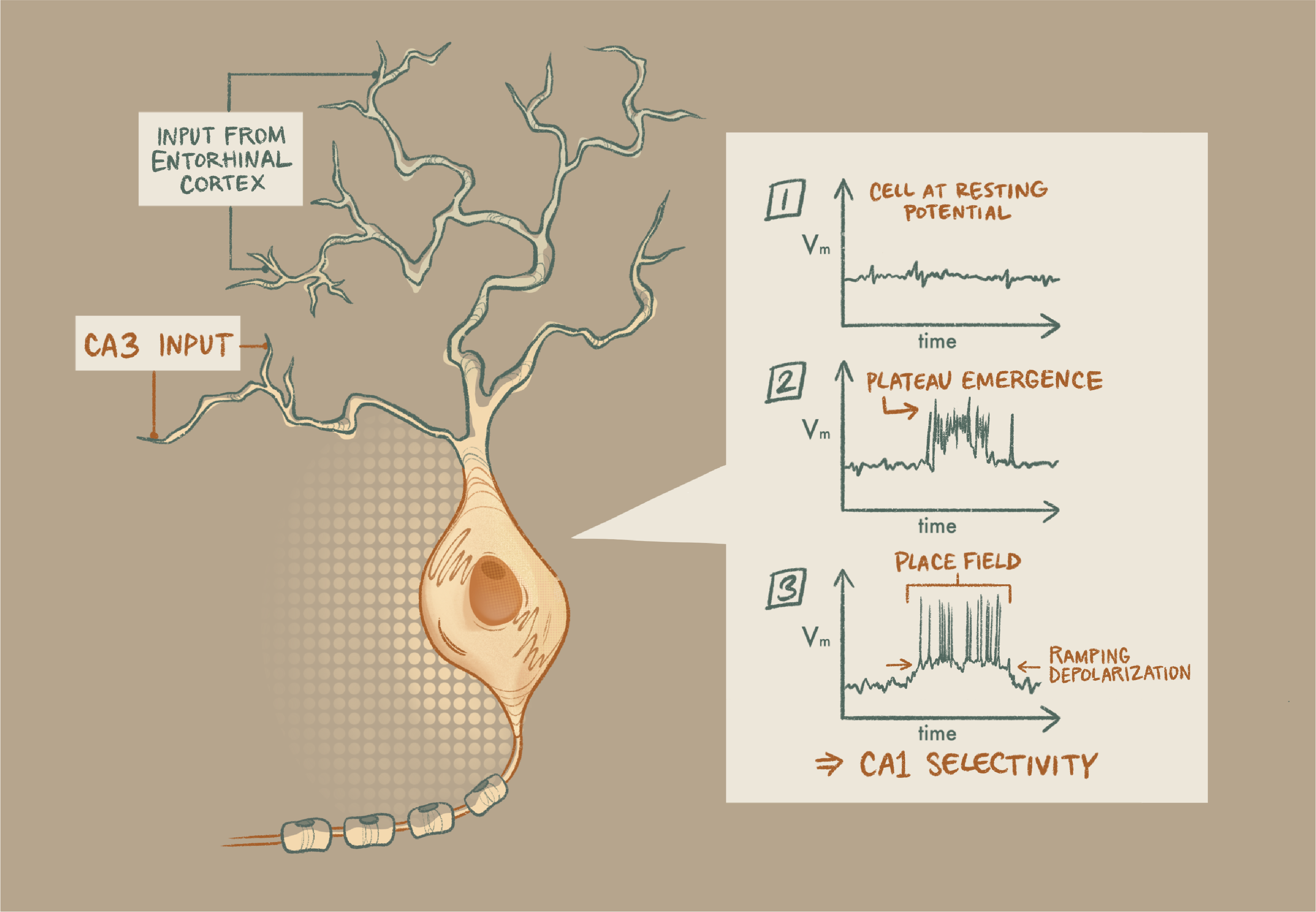
Working through the figures
One wonderful feature of this paper’s organization is that each figure corresponds to a particular window into these longer timescales of cellular memory, each covering a useful technique in neuroscience: in vivo electrophysiology and behavior, modeling, in vitro slice physiology and pharmacology.
Figure 1 shows the very first observation of BTSP. While recording intracellularly from CA1 hippocampal neurons in a mouse running on a treadmill, the authors noticed a place field spontaneously appear in a silent neuron. Figure 1A and 1B show the development of this place field, first as a heatmap and then as single voltage traces. Although this example neuron was silent through the first 10 trials, it started spontaneously spiking in a particular area of the track on trial 11, replicating their previous findings.
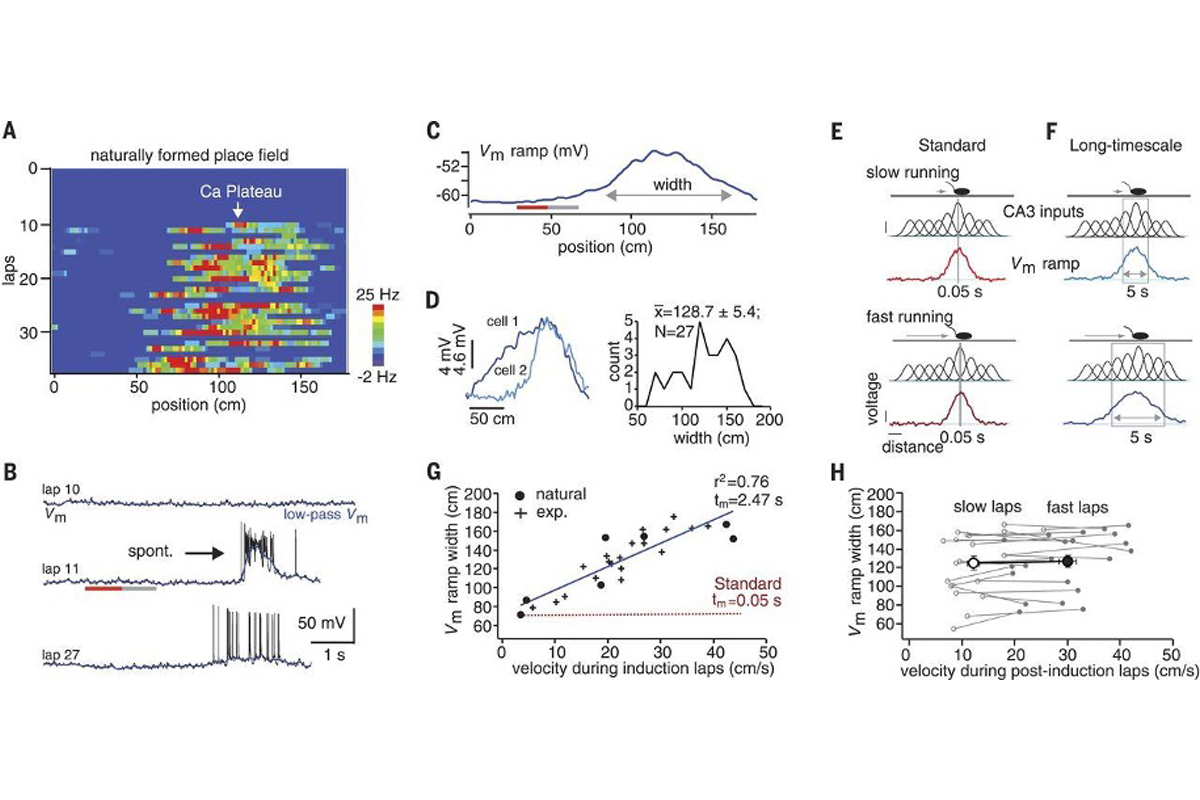
The first half of this figure should be straightforward for students: The first four panels of the figure contain linear place fields along with voltage traces from their neurons. It is worth noting that the researchers filtered their traces to remove spiking activity and isolate the slower changes in the signal—the long-lasting depolarizations that persist in the cells. They found that both naturally occurring and experimentally induced (via stimulation) plateau potentials caused a measurable depolarization of the membrane and the initiation of a place field. Interestingly, you can find a subthreshold signature of a place field earlier (in time and space) than the place where the plateau was evoked, suggesting that this plasticity begins seconds earlier.
The second half of Figure 1 is a little trickier to parse. Keeping in mind that mice are running on a treadmill, and that place fields are already formed in upstream hippocampal area CA3, the authors show that the size of the induced place field size depends on how fast the animal is running during the trial. Understanding why this is the case may furrow your brow a bit (it did mine, at least). Essentially, because CA1 is inheriting place fields from CA3, more cells from CA3 fire when the mouse covers more distance. More strengthened inputs over longer distances (which you can see illustrated as lots of Gaussian bumps underneath the mouse in 1E and 1F) leads to longer voltage ramps.
In Figure 2, the authors used modeling to determine whether their data demonstrate typical Hebbian plasticity (within milliseconds) or longer plasticity. You could skip this figure altogether if you’re not interested in dissecting the model; the take-home message is this: When you use CA3 place-field inputs based on the animal’s movement, you can model the CA1 data and estimate a time frame over which plasticity occurs. Importantly, the model showed that plasticity could arise seconds before and after the initiation of a plateau potential, a much longer timescale than Hebb’s rule.
In Figure 3, the authors used electrical stimulation in brain slices to see if they could observe this plasticity while precisely controlling the timing of both the pre- and post-synaptic activity. By varying the timing of the stimulation of CA3 axons or input into the CA1 cell itself to generate plateau potentials, they showed that they could increase synaptic strength even when the plateau potential was initiated seconds before or after the CA3 axons were stimulated. Remarkably, and also in opposition to Hebb’s rule, they could do this with just five electrical stimulations. The data in Figure 3D nicely mirror Figure 2G, demonstrating how modeling and experimental work can go hand in hand.
Finally, the authors used pharmacological approaches to demonstrate that it really, truly is the plateau potentials that drive plasticity. Magee’s group had previously shown that NMDA receptors and Ca+2 channels are essential for dendritic plateaus. In the 2017 paper, they showed that blocking either prevents the increase in synaptic strength and that blocking Ca+2 channels in vivo stops formation of place fields in CA1 neurons.
Sticky points
The bulk of this paper is a nice tour of techniques that are commonly covered in undergraduate neuroscience classes. However, many of the experimental details are a bit buried (this is a Science paper, after all). For example, it’s not immediately clear that Figure 4 contains both slice and in vivo experiments. You may choose to focus on the conceptual findings, but personally I think it is just as important to really drill down into how they did these experiments, especially so that students can appreciate these experiments’ technical difficulty.
The scientists behind the paper
Katie Bittner, now a clinical program manager at Medtronic, joined Magee’s lab as a postdoctoral fellow. At the time, the lab was primarily focused on the cellular mechanisms of dendritic processing, she says. But she and others noticed a gap in the field: Researchers studying brain slices could demonstrate the different computations neurons could perform, but they hadn’t yet determined what computations were most important for behavior. Bittner also says that the ability to record from single cells in awake-behaving animals—which experimentalists were just starting to do—was essential to be able to address these kinds of questions. “In order for us to get at these mechanisms, we really needed access to the cell in a way that people hadn’t really done before,” Bittner says.
Bittner says the experiments worked surprisingly well, so well that she was immediately skeptical and asked another member of the lab to try to replicate it. “We did a lot to be like, ‘Is it an artifact? Is it something with the animal’s behavior?’ Just to really make sure it was a robust phenomenon,” Bittner says.
Then-postdoc Aaron Milstein, now assistant professor of neuroscience and cell biology at Rutgers University, led the modeling efforts of the paper, which seemed baffling at first. “The model was spitting out this stuff that looked like garbage,” Milstein says. “The precise spike timing [didn’t] seem to matter at all.” So they dug into the data even further and ran additional in silico and in vitro experiments to finally confirm that yes, plasticity was happening over unpreceded timescales.
Additional reading
Since this 2017 paper was published, Magee’s group and others—for example, Fan et al. (2023) and Gonzales et al. (2023), which both use a fully optical rather than an electrophysiological approach to these questions—have illustrated, using a range of techniques, that these findings are robust. Neuroscientist Peter Rupprecht has compiled a useful summary of these papers. Other groups are using these findings to artificially induce place cells so that they can study their properties. Now heading his own lab, Milstein is investigating the role of dendritic plateaus as a sort of top-down “teaching” signal from cortex, trying to figure out what drives plateau potentials in behaving animals. For additional content, students can watch Magee’s 2023 lecture at the McGovern Institute, in which he discusses the body of work that followed from this paper.
Recommended reading
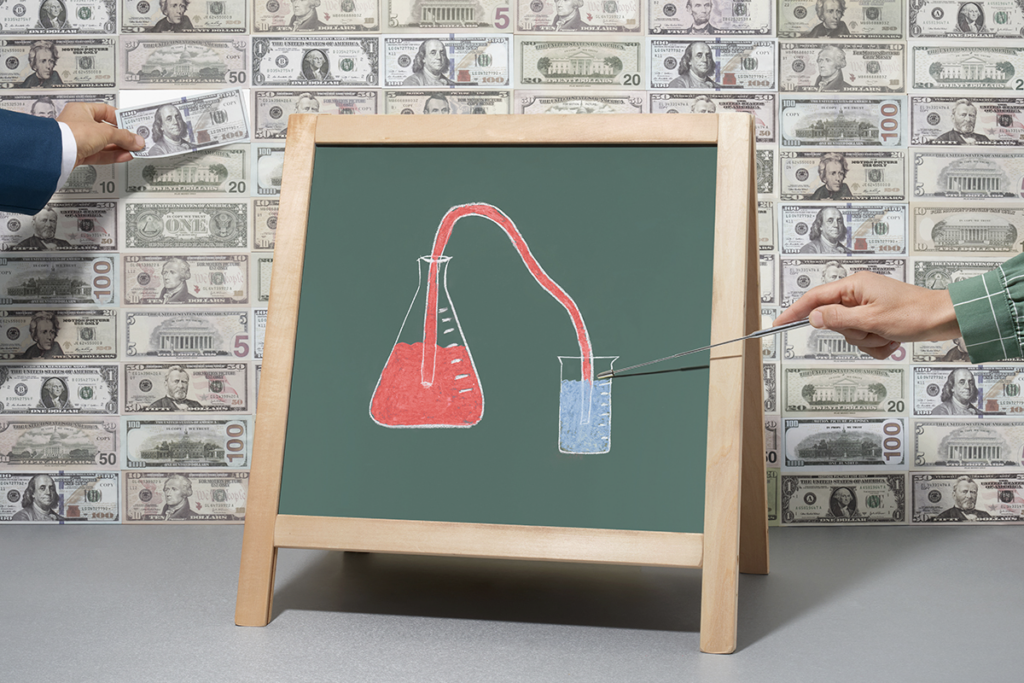